Exploring the Sanger Sequencing Methodology
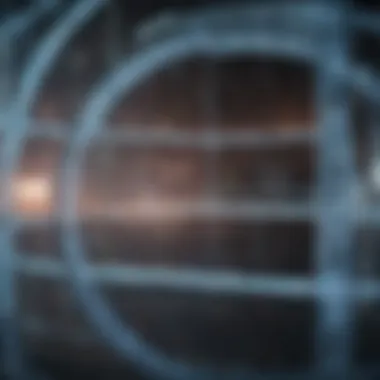
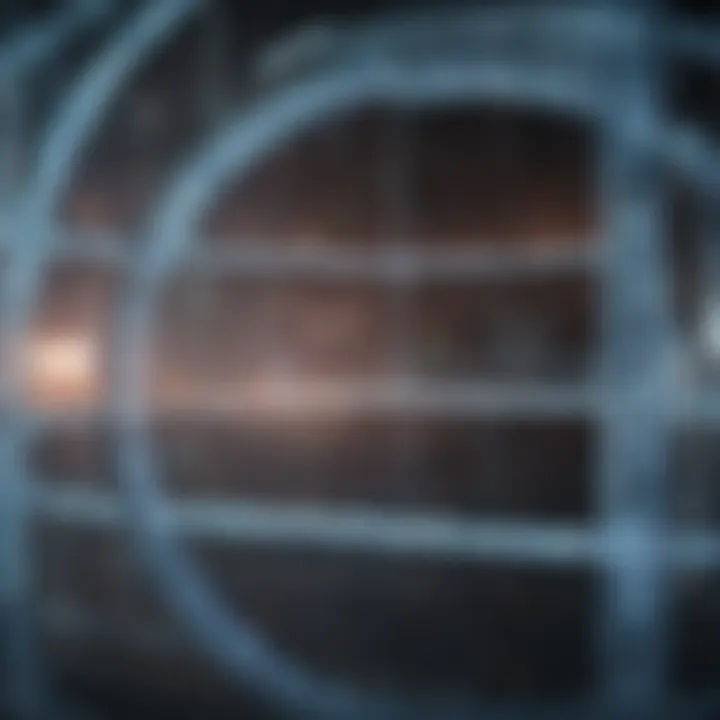
Intro
Sanger sequencing, developed by Frederick Sanger in the 1970s, stands as a pivotal technology in molecular biology. This method allows for the determination of nucleotide sequences in DNA, making it pivotal for many genomic studies. It combines simplicity with reliability, and remains relevant even in an age marked by rapid advancements in sequencing technologies. Understanding this process provides insight not only into how information is gleaned from genetic materials, but also into its broad applications in research and medicine. In the following sections, we will elaborate on the methodology of Sanger sequencing and its relevance to genetic research.
Methodology
Sanger sequencing employs a straightforward yet sophisticated process. This section will break down its core elements, including the experimental framework and the techniques utilized during data acquisition.
Study Design
The Sanger sequencing method is based on the principle of selective incorporation of chain-terminating dideoxynucleotides during DNA replication. It involves the original DNA strand, which serves as a template for synthesis. Importantly, for Sanger sequencing to work, it requires a DNA polymerase enzyme, primers, as well as four types of regular deoxynucleotides (dATP, dGTP, dCTP, dTTP) and modified dideoxynucleotides (ddATP, ddGTP, ddCTP, ddTTP). Each of these dideoxynucleotides is fluorescently labeled for detection.
Data Collection Techniques
The next step involves the synthesis of new DNA strands. As the process continues, incorporation of a dideoxynucleotide will terminate the elongation of that particular strand, resulting in DNA fragments of different lengths. Post-reaction, capillary electrophoresis is employed to separate these fragments based on their size. As each fragment emerges, it is detected to determine its sequence based on the corresponding fluorescent label. Thus, this system yields a sequence readout, providing critical genetic information.
"Sanger sequencing has been essential for many areas of genetics including diagnostics, forensics, and biological research."
Discussion
The Sanger sequencing process not only produces accurate data but also lays a foundational methodology upon which more advanced sequencing technologies are built. This section shall delve into the interpretation of results derived from Sanger sequencing, its limitations, and possible future research trajectories.
Interpretation of Results
Data output from Sanger sequencing presents distinct peaks, allowing researchers to identify the order of nucleotides efficiently. Each peak corresponds to a specific nucleotide, thereby enabling the construction of a readable sequence.
Limitations of the Study
Despite its accuracy, Sanger sequencing has limitations. These include a relatively low throughput compared to next-generation sequencing technologies. It may also struggle with complex regions of DNA that are repetitive or highly variable.
Future Research Directions
Future research can focus on enhancing Sanger sequencing methods, integrating them with other technologies, and addressing present limitations. The goal is to maintain relevance in the ever-evolving field of genetics. As we continue to grapple with complex genetic issues, refining Sanger sequencing could play a vital role in clinical diagnostics, genetic research, and precision medicine.
Understanding the foundational aspects of Sanger sequencing illuminates its ongoing significance within molecular biology, illustrating why its principles persist even alongside newer technologies.
Preamble to Sanger Sequencing
Sanger sequencing, a pivotal method in molecular biology, has profoundly shaped our comprehension of genetics. Its importance extends beyond mere DNA analysis; it serves as a gateway to understanding genetic variation, disease mechanisms, and evolutionary relationships among organisms. This section will delve into the history and innovations that define Sanger sequencing, illustrating its enduring relevance in contemporary biological research and diagnostic practices.
Origins of the Method
The development of Sanger sequencing traces back to the early 1970s. At that time, the race to decode the genetic makeup of organisms was gaining momentum. The method was pioneered by Frederick Sanger, a British biochemist, who sought an efficient means to determine the precise sequence of nucleotides in DNA.
The original method, known as the dideoxy chain termination method, introduced groundbreaking concepts that addressed the limitations of previous sequencing techniques. Sanger's approach not only provided a systematic framework for sequencing but also emphasized the importance of accuracy, which is essential for any genetic analysis.
Moreover, Sanger sequencing was firt recognized after its application in sequencing the genome of the virus MS2 in 1976, establishing itself as a reliable tool for genetic research. Over the decades, this method has been incorporated into numerous studies, from determining human genetic disorders to exploring microbial diversity.
Key Innovations by Frederick Sanger
Frederick Sanger's contributions to the field of genetics are noteworthy. His key innovations that shaped Sanger sequencing include:
- Dideoxynucleotides: Sanger introduced modified nucleotides known as dideoxynucleotides, which lack a 3'-OH group. This simple change created a mechanism for terminating DNA strand synthesis, allowing for more precise determination of DNA sequences.
- Labeling Methods: Sanger's incorporation of fluorescent dye labeling enabled the visualization of sequencing products. This advancement simplified the subsequent analysis of DNA fragments and facilitated greater throughput.
"The development of dideoxy chain termination was a game changer that made DNA sequencing far more efficient and reliable."
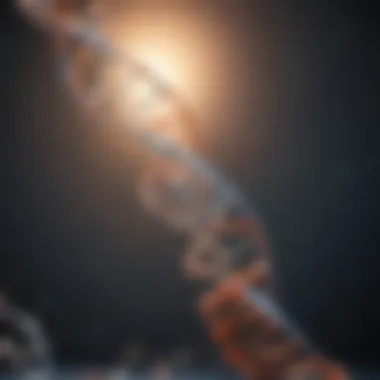
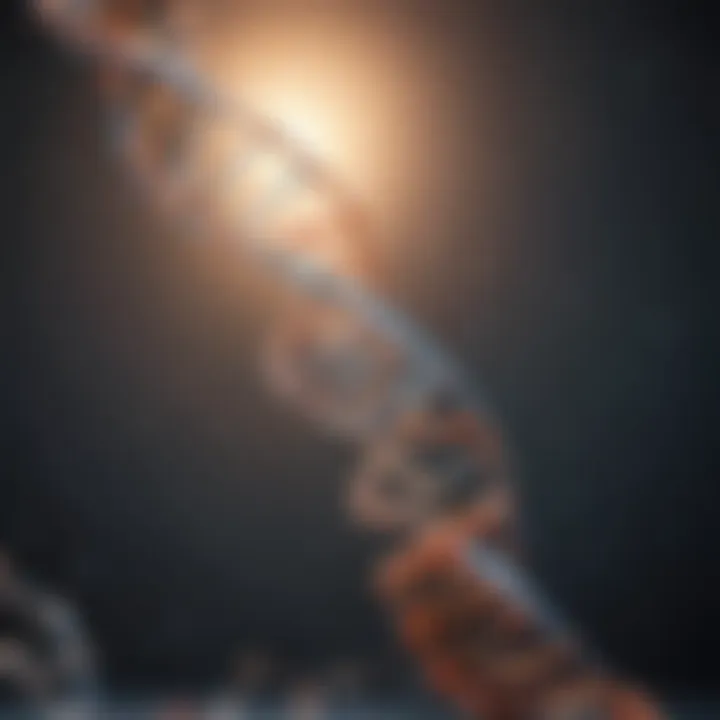
- Gel Electrophoresis: The utilization of gel electrophoresis for separation of DNA fragments was fundamental in identifying the length and thus the sequence of the nucleotides. This technique significantly improved the accuracy and reliability of the sequencing process.
These innovations remain integral to many DNA sequencing methodologies today. From its initial conception to its adoption in high-throughput sequencing, Sanger sequencing has laid the groundwork for advancements in genomics and biotechnology. Its impact continues to inform new research approaches and clinical applications.
Fundamental Principles of Sanger Sequencing
The Sanger sequencing method, developed by Frederick Sanger in the 1970s, relies on specific foundational principles essential for accurate DNA sequencing. Understanding these principles is vital, as they explain how Sanger sequencing remains a standard technique in molecular biology today. Key elements include the basic conceptual framework, the role of DNA polymerase, and the importance of chain termination. These aspects not only underline the method's scientific significance but also contribute to its applications in various fields such as genetics and diagnostics.
Basic Conceptual Framework
The fundamental framework of Sanger sequencing is based on the synthesis of DNA strands. In this process, a single-stranded DNA template serves as a guide for synthesizing a complementary strand. The system uses a limited amount of dideoxynucleotides, which are crucial for terminating the DNA chain. Understanding this framework helps to appreciate the balance between DNA replication and termination, which is pivotal for obtaining accurate sequence data. Essentially, the procedure can be summarized as:
- Preparation of the template: Single-stranded DNA is obtained and purified.
- Extension of the strand: DNA polymerase synthesizes new DNA by adding nucleotides.
- Termination: The incorporation of dideoxynucleotides leads to chain termination, producing fragments of varying lengths.
Role of DNA Polymerase
DNA polymerase is one of the most critical enzymes in the Sanger sequencing process. This enzyme is responsible for catalyzing the addition of nucleotides complementary to the DNA template. It works efficiently in extending the growing DNA strand. The activity of DNA polymerase is highly specific; it recognizes the correct base pairs and incorporates the nucleotides into the DNA strand.
In Sanger sequencing, the enzyme operates under a controlled environment, where both deoxynucleotides and dideoxynucleotides are present. This mixture ensures a fine balance between normal chain extension and the eventual termination of the sequence. Without the precise action of DNA polymerase, the sequencing process would not yield reliable results, indicating its fundamental role in the methodology.
Importance of Chain Termination
Chain termination is a defining feature of Sanger sequencing. The process introduces dideoxynucleotides, which lack a necessary hydroxyl group needed for further elongation of the DNA chain. By incorporating these modified nucleotides, DNA synthesis is halted at specific points, resulting in fragments whose lengths correspond to the location of the terminated sequences.
This controlled termination allows researchers to determine the sequence of the original DNA template. The generated fragments are then separated by size and analyzed to read the sequences of nucleotides, revealing important genetic information. Overall, the principle of chain termination not only enhances the accuracy of Sanger sequencing but also differentiates it from other sequencing methods.
Chain termination using dideoxynucleotides is what allows for precise identification of nucleotides in DNA sequences.
In summary, the fundamental principles of Sanger sequencing establish the scientific basis for the technique. A clear understanding of the basic conceptual framework, the role of DNA polymerase, and the importance of chain termination allows for a deeper appreciation of its applications, particularly in genetic testing, research, and diagnostics.
Step-by-Step Sanger Sequencing Protocol
The Step-by-Step Sanger Sequencing Protocol is crucial in understanding how this method operates efficiently for DNA sequencing. This section provides a detailed examination of each step involved in the process, emphasizing the precise actions taken to ensure reliable results. The objective is to underscore the careful preparations, specific reagents used, and analytical methods that characterize Sanger sequencing. Understanding these steps is essential for students and professionals wishing to implement or study sequencing techniques.
Preparation of the DNA Template
The preparation of the DNA template is the first and vital step in the Sanger sequencing process. This step involves isolating and purifying the segment of DNA that will be sequenced. Typically, this is done through methods like polymerase chain reaction (PCR), which amplifies targeted DNA sequences to create enough material for sequencing.
Moreover, the quality and integrity of the DNA template influence the overall accuracy of the sequencing results. It is important to avoid contamination and ensure the template is intact. The purity of the DNA can be assessed using spectrophotometry, ensuring that the ratios of absorbance are within acceptable ranges. A well-prepared DNA template is essential as it lays the foundation for subsequent steps.
Incorporation of Dideoxynucleotides
The incorporation of dideoxynucleotides (ddNTPs) is a defining feature of the Sanger method. In this step, four distinct reaction mixtures are prepared, each containing normal deoxynucleotides and one type of ddNTP. The key property of ddNTPs is that they lack a hydroxyl group at the 3' position, preventing further elongation of the DNA chain, thus causing chain termination.
When DNA polymerase extends the primer with ddNTPs, the process results in fragments of varying lengths that are dependent on where the ddNTPs are incorporated. This results in a series of labelled DNA fragments, each ending with a specific base. The ratio of ddNTPs to regular deoxynucleotides is critical and must be optimized to ensure a balanced mix of fragment sizes.
Amplification of DNA Fragments
Once ddNTPs are incorporated, the next step is amplifying the resultant DNA fragments. This amplification can be achieved by employing thermal cycling, which consists of repeated heating and cooling. These cycles promote denaturation, annealing of primers, and elongation by DNA polymerase. Each cycle effectively doubles the amount of DNA, leading to abundant sequencing products.
Rapidly, these amplification steps accumulate millions of copies of the original DNA sequences. The use of specific primers designed to target homologous regions is crucial for ensuring the accuracy and specificity of the amplified products. Without proper amplification, the remaining sequencing steps may yield insufficient data for reliable analysis.
Separation of Sequencing Products
After amplification, the molecules need to be separated based on size to interpret the sequence properly. This separation typically occurs through capillary electrophoresis or gel electrophoresis. In capillary electrophoresis, the DNA fragments are propelled through a gel matrix at different rates depending on their sizes.
Smaller fragments move faster than larger ones, allowing for the resolution of sequences. Each fragment is then detected as it passes through a laser, and the emitted fluorescence is recorded, generating a readout detectable as a chromatogram. This separation is crucial, as it enables the identification of the terminated fragments, which determine the DNA sequence.
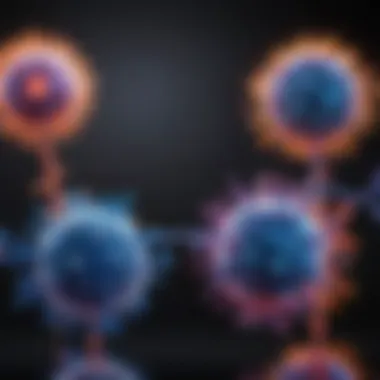
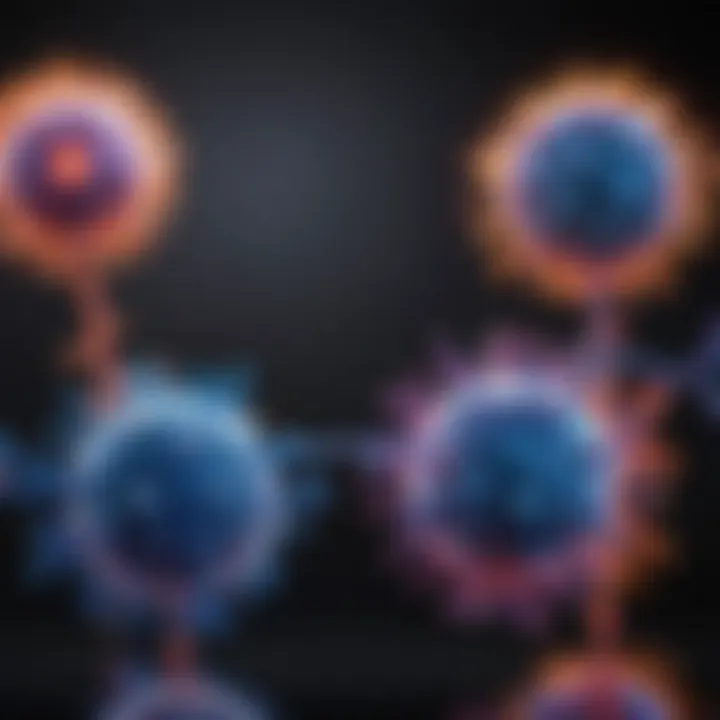
Reading the Sequence Data
The final step involves interpreting and reading the sequenced data. This is accomplished by analyzing the chromatogram generated in the previous step. Each color of fluorescence corresponds to a specific base, revealing the sequence of nucleotides.
Software tools can be used to automate the readout process, facilitating the alignment of sequences and error-checking. Furthermore, validation of results is important in ensuring that the sequenced data is accurate and reliable. Typically, multiple independent sequencing runs are conducted to confirm results.
In summary, recognizing each phase within the Sanger sequencing protocol is essential. The method's systematic approach allows for reliable and precise genome analysis. Understanding it equips professionals to employ Sanger sequencing effectively for various applications in genetic testing, diagnostics, and molecular biology.
Applications of Sanger Sequencing
Sanger sequencing remains a cornerstone technique in molecular biology for various applications. Its precision and reliability make it a preferred method in many settings, particularly where accuracy is paramount. This section will delve into the specific applications of Sanger sequencing, focusing on genetic testing, research, and clinical usage. Understanding these applications is crucial for recognizing how this method continues to shape the landscape of genetic research and diagnostics.
Genetic Testing and Diagnostics
Sanger sequencing plays a vital role in genetic testing and diagnostics. It is frequently used to identify mutations linked with hereditary diseases. For example, it can pinpoint specific genetic alterations in conditions like cystic fibrosis or Huntington's disease. The accuracy provided by Sanger sequencing is critical when determining the presence of pathogenic variants.
Many laboratories rely on this technology to produce definitive results that can guide treatment options. In prenatal testing, Sanger sequencing helps in identifying genetic disorders in fetuses. Its high accuracy rate provides expecting parents with reliable information.
Sanger sequencing has become the gold standard in genetic diagnostics due to its accuracy and reliability.
Research in Molecular Biology
In the research landscape, Sanger sequencing facilitates a wide range of molecular biology studies. It allows scientists to determine the nucleotide sequences of DNA, playing a pivotal role in genomics and evolutionary biology. Researchers often utilize this technique for clone verification when studying gene function or mutation effects. Sequencing plasmids or PCR products is common practice, enabling confirmation of insert sequences in various applications.
Additionally, Sanger sequencing is crucial in evolutionary studies, helping trace lineage and the evolution of species. By providing a reliable method to sequence DNA regions, it contributes to insights into genetic diversity and adaptation. Through generating ample sequence data, a better understanding of phylogenetic relationships emerges.
Clinical Applications
Clinical applications of Sanger sequencing extend beyond diagnostics. This method is used in various therapeutic contexts, particularly concerning cancer genetics. Identifying mutations in cancer-related genes can guide personalized treatments. For instance, certain mutations in the BRCA1 or BRCA2 genes signal increased cancer risks and influence patient management strategies.
Sanger sequencing also aids in developing targeted therapies, allowing healthcare professionals to tailor treatments that align with individual patient biology. Furthermore, in microbiology, Sanger sequencing identifies pathogenic organisms through precise sequencing of their genetic material.
Advantages and Limitations of Sanger Sequencing
Evaluating the advantages and limitations of Sanger sequencing is essential for understanding its current applications and relevance in modern genomics. This method has been a cornerstone in genetic research for decades, but it has both strengths and drawbacks that need to be acknowledged. With the rise of alternative sequencing technologies, knowing the advantages and limitations becomes more crucial for researchers, educators, and students alike.
Benefits of High Accuracy
Sanger sequencing is known for its high accuracy in determining DNA sequences. The method employs chain-terminating dideoxynucleotides, which ensure that errors in base pairing are minimal. The process yields sequences that have an accuracy rate often exceeding 99%. This level of precision is vital in applications where genetic integrity matters, such as clinical diagnostics and SNP analysis.
Moreover, the ability to produce clean and clear chromatograms means researchers can visualize the sequencing results effectively. Such visual data support the detection of errors or anomalies in the sequence data right away. In a world where inaccurate data can lead to misleading conclusions, the reliability of Sanger sequencing stands out.
Cost Considerations
When it comes to cost, Sanger sequencing is frequently seen as more expensive compared to next-generation sequencing (NGS) methods. While the price of whole genome sequencing continues to drop, the per-sample cost of Sanger sequencing often remains high due to the reagents and processes involved.
However, the specific context can alter the cost patterns. For low-throughput applications, such as validating specific genetic sequences, Sanger can actually be more economical. Researchers must evaluate whether their project is suited for Sanger and understand that while initial investment might be high, the insights gained could outweigh these costs, especially when high accuracy is a priority.
Limitations in Throughput
One of the most notable limitations of Sanger sequencing is its throughput capability. The method is inherently limited to producing a smaller amount of data at any given time. Typically, it can sequence DNA fragments of about 800 to 1000 base pairs, making it challenging to apply for large-scale projects where extensive sequencing is required.
This constraint means that, for large genomes or expansive genomic regions, Sanger sequencing is not practical. In contrast, technologies like Illumina and PacBio sequencing can generate millions of sequences in parallel, drastically increasing efficiency. Thus, while Sanger remains a robust method for targeted applications, its throughput limitations often necessitate using alternative methods for broader sequencing tasks.
Comparison with Next-Generation Sequencing
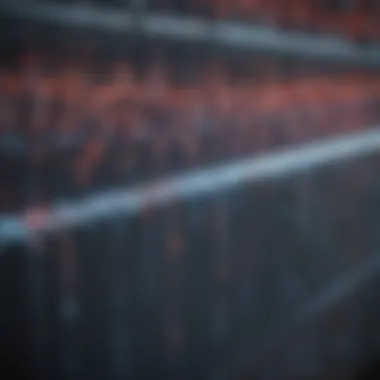
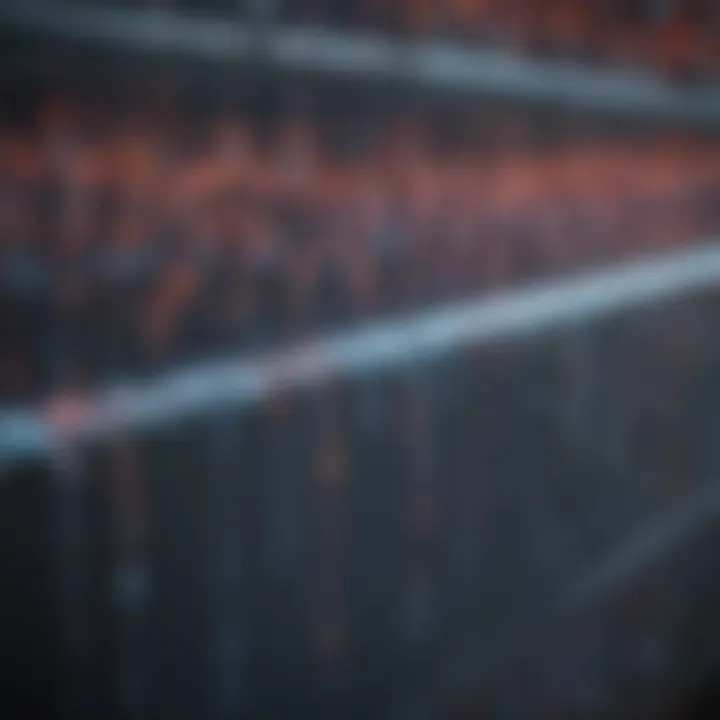
The comparison between Sanger sequencing and Next-Generation Sequencing (NGS) is critical to understanding the evolution of genetic analyses. Each of these methods has its own set of strengths and weaknesses, catering to different needs in the research and clinical environments. Analyzing these differences allows for enhanced appreciation of their respective roles in modern genomics. This section will elucidate key methodological differences, data outputs, interpretation variations, and specific use cases.
Differences in Methodology
Sanger sequencing and NGS employ distinct approaches to decipher genetic sequences. Sanger sequencing relies on the chain termination method, where dideoxynucleotides are incorporated into the growing DNA strand, leading to fragment termination. This results in a limited number of reads processed at one time, usually around 384 samples in a single run. Conversely, NGS applies massively parallel sequencing technologies, allowing for millions of short sequences to be generated simultaneously.
Some fundamental differences include:
- Read Length: Sanger sequencing typically yields longer reads, commonly 500 to 1000 base pairs, while NGS usually generates shorter reads, around 50 to 300 base pairs.
- Throughput: Sanger sequencing has lower throughput compared to NGS due to its sequential processing nature. NGS provides high throughput by processing multiple fragments in parallel.
- Costs: In terms of cost per base, Sanger is generally more expensive than NGS due to the lower throughput and higher consumable costs associated with each reaction.
Data Output and Interpretation
The output of Sanger sequencing is comparatively straightforward. It produces a sequence read that can be interpreted directly. Each run produces a clean chromatogram that depicts the DNA sequence visually. This clarity provides a high level of confidence in the generated data.
On the other hand, NGS data can be complex. Each sequencing run can yield vast amounts of data, with millions of short sequences generated. This necessitates the use of bioinformatics tools for data assembly and alignment. Interpreting NGS results may involve more sophisticated analytical pipelines to ensure accuracy and relevance in the results.
"The understanding of sequencing technologies, especially Sanger and NGS, is vital for anyone involved in genomic research. Each method offers unique advantages that should be matched with specific project needs."
Use Cases for Both Technologies
When deciding which sequencing method to utilize, one must consider the specific application.
- Sanger Sequencing: This method is preferred for smaller scale projects, like individual gene sequencing, validation of NGS results, and in clinical settings for targeted sequencing. It offers precise data and lower error rates for such applications.
- Next-Generation Sequencing: NGS excels in large-scale applications such as whole genome sequencing, transcriptome analysis, and high-throughput genotyping. Its ability to generate vast amounts of data in parallel makes it suitable for extensive genomic projects.
In summary, while both Sanger sequencing and NGS have their own merits, the choice between them often hinges on project scale, needed accuracy, and budget. Understanding the capabilities of each method will better equip researchers and clinicians in their genetic analysis endeavors.
Future Perspectives of Sanger Sequencing
The landscape of genetic sequencing is evolving rapidly due to technological advancements. Within this context, Sanger sequencing maintains a significant role. However, its future depends on innovations and integrations that enhance its utility. Technology continues to develop, prompting searches for improved accuracy, speed, and cost-effectiveness. This section explores potential innovations and how Sanger sequencing can integrate with various modern technologies to maintain its relevance in the field of genetics.
Innovations in Methodology
The Sanger sequencing methodology is not stagnant. Researchers continually refine techniques to enhance its performance. Some possible innovations include the following:
- Automated Systems: Automation has already improved efficiency and reduced human error. Advancements will likely lead to systems that require minimal oversight.
- Improved Dideoxynucleotides: The development of more efficient dideoxynucleotides could improve sequencing speed and accuracy. By optimizing the chemical properties of these nucleotides, researchers can minimize failures in incorporating them into sequences.
- Size-Selective Gel Electrophoresis: Enhancements in gel electrophoresis methods could yield better resolution of DNA fragments, leading to more precise sequence reads.
- Real-Time Sequencing Techniques: Pairing real-time data analysis with Sanger sequencing can allow researchers to observe reactions as they occur. This could accelerate data acquisition and interpretation, making the process even more user-friendly.
Such innovations signify that Sanger sequencing is adapting to meet contemporary scientific demands. As these improvements find their way into mainstream use, Sanger sequencing might retain its place as a reliable method among newer technologies.
Integration with Other Technologies
To remain pertinent, Sanger sequencing will need to integrate with emerging technologies. This process can enhance its capabilities and extend its applications. Possible integrative approaches include:
- Next-Generation Sequencing (NGS): By combining Sanger sequencing with NGS, researchers could leverage the strengths of both. While NGS allows for high-throughput sequencing, Sanger sequencing could provide confirmation of critical findings due to its high accuracy.
- Bioinformatics Tools: Incorporating advanced bioinformatics will enable more sophisticated data analysis. This can streamline the process of interpreting large datasets and enhance accuracy in variant detection.
- CRISPR Technology: The combination of Sanger sequencing with CRISPR techniques could facilitate precise edits based on exact sequences, making targeted gene therapy more viable.
The integration of new technologies into Sanger sequencing highlights the adaptability of this foundational method in response to the rapidly evolving genetic landscape.
The integration of such technologies will broaden the scope of Sanger sequencing applications. The capacity for reliability remains essential as the genetic field continues to change.
Epilogue
The conclusion of this article underscores the significance of Sanger sequencing within the broader context of molecular biology. Sanger sequencing is not only a historical milestone but also a technique that remains relevant in various scientific domains today.
Summary of Key Points
- Historical Context: Sanger sequencing originated from the groundbreaking work of Frederick Sanger in the 1970s, establishing a standard for genetic analysis.
- Methodological Importance: The technique relies on DNA polymerase and dideoxynucleotides to achieve high accuracy in sequencing, ensuring reliable results for genetic testing.
- Wide Applications: Sanger sequencing has practical applications in genetic testing, research, and clinical diagnostics, making it essential in the field of genetics.
- Future Outlook: While newer technologies are evolving, Sanger sequencing retains its value in specific use cases, particularly where precision is critical.
The depth of this article also highlights the method's advantages, such as its accuracy and reliability compared to emerging alternatives. These elements motivate researchers and professionals to continue utilizing Sanger sequencing in various contexts.
The Enduring Value of Sanger Sequencing
Sanger sequencing serves as a cornerstone technique in our understanding of genetics. Its continued application in genetic testing and research illustrates its ongoing relevance. This method offers benefits, including:
- High Accuracy: Sanger sequencing provides precise data that researchers can trust for critical analyses.
- Established Protocols: Decades of refinement have created standardized procedures that many labs utilize worldwide.
- Integration with New Technologies: The technique is increasingly being combined with next-generation sequencing for specific applications.