Converting DNA Sequences to RNA Sequences
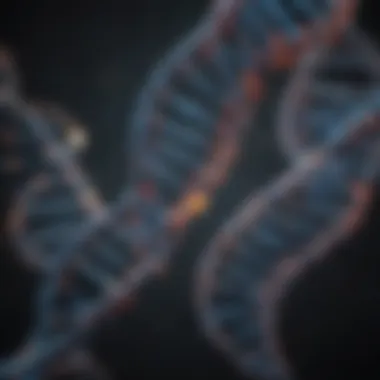
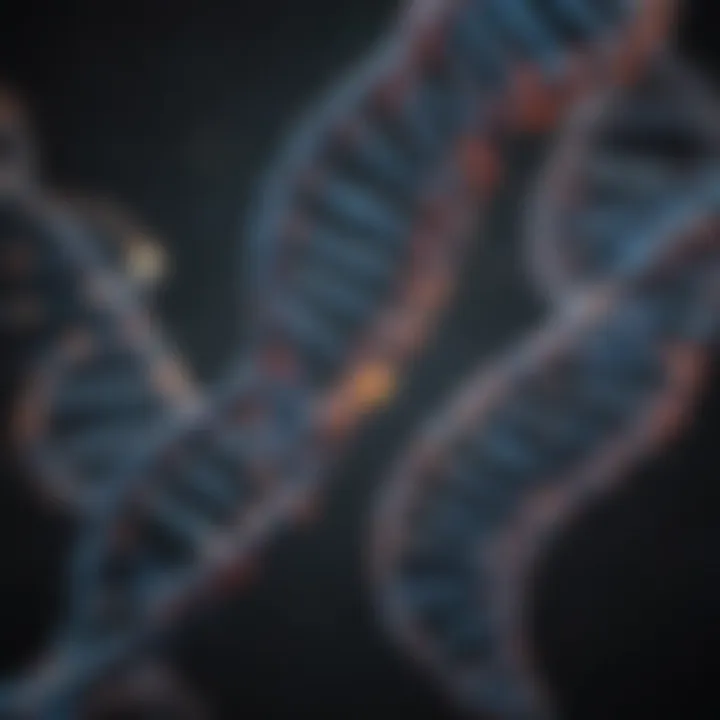
Intro
In the realm of molecular biology, the conversion of DNA sequences to RNA sequences is a fundamental process. This topic not only underpins essential biological functions but also contributes to numerous biotechnological advancements. Understanding this conversion process provides insights into how genes are expressed and regulated, which is crucial for students, researchers, and professionals alike. This comprehensive guide aims to demystify the mechanisms involved, from the principles of transcription to the various tools available for accurate conversions.
Methodology
Study Design
The methodologies for converting DNA to RNA vary widely and depend on the specific applications or experiments being conducted. It is important to employ rigorous study designs to ensure accuracy and reliability. Researchers may choose experimental techniques or computational models for conversion based on their particular focus. The most prevalent methods include in vitro transcription and bioinformatics tools designed for sequence conversion.
Data Collection Techniques
Data collection plays a vital role in the conversion of DNA sequences. For instance, sequencing technologies like Sanger sequencing or Next-Generation Sequencing (NGS) yield raw DNA data which can then be translated into RNA sequences. In silico methods also utilize databases and algorithms that rely on pre-existing data to infer RNA sequences from given DNA strands. Both approaches emphasize the importance of accurate data in ensuring successful conversion.
Discussion
Interpretation of Results
Interpreting the results of DNA to RNA conversion is not straightforward. The accuracy of the results can hang on the methods used in study design and data collection. A high fidelity in transcription is critical for applications such as gene expression analysis and functional genomics. Anomalies in this process can lead to misrepresentation of biological functions, impacting downstream applications.
Limitations of the Study
While the study of converting DNA to RNA is well-established, several limitations persist. For one, the accuracy of computational predictions can be influenced by sequence complexity and biological noise. Furthermore, some methods may not capture alternative splicing events adequately, which are crucial in understanding gene function.
Future Research Directions
Looking ahead, there is significant potential to improve DNA to RNA conversion techniques. Advances in algorithms could enhance the predictive capabilities for more complex biological systems. Additionally, integrating machine learning may further refine the conversion accuracy, providing researchers with better tools for genetic analysis. Exploring these avenues could greatly enrich our understanding of genetics and its applications in biotechnology.
"The journey from DNA to RNA is a voyage through the complexity of life itself."
Foreword to DNA and RNA
In the realm of molecular biology, understanding the roles and differences between DNA and RNA is foundational. This section lays the groundwork for appreciating how DNA sequences are converted to RNA sequences. Grasping these concepts is crucial, not only for students but also for researchers and educators who delve into genetic studies and applications.
A basic but solid comprehension of DNA and RNA helps illuminate the biological significance of the transcription process. Transcription is essential for protein synthesis, gene expression, and ultimately, the function of living organisms. Without this knowledge, the intricate processes of life may seem chaotic or simplistic. By unraveling the complexities of DNA and RNA, we can better appreciate their involvement in genetic manipulation and biotechnology, expanding our insight into genetic research.
Fundamental Differences Between DNA and RNA
DNA and RNA, though similar in some aspects, exhibit key differences that are paramount in their functions. DNA, or deoxyribonucleic acid, is typically double-stranded and serves as the template for genetic information. RNA, or ribonucleic acid, is usually single-stranded and plays a role in translating that genetic information into proteins.
Key differences include:
- Structure: DNA has a double helix structure, while RNA generally forms a single strand.
- Sugar Component: The sugar in DNA is deoxyribose, compared to ribose in RNA.
- Nitrogenous Bases: DNA uses thymine, whereas RNA substitutes it with uracil. This small change greatly influences how these molecules function.
These distinctions are essential for understanding how DNA is transcribed into RNA and how this process is critical for protein synthesis.
Structural Components of DNA and RNA
The structural components of DNA and RNA contribute significantly to their function. DNA consists of long chains of nucleotides, which are made up of a phosphate group, a sugar, and nitrogenous bases. The sequence of these bases encodes genetic information.
In contrast, RNA's structure is more versatile. It also consists of nucleotides, but its generally shorter chain allows for a variety of forms, such as mRNA, tRNA, and rRNA, each serving different purposes within the cell.
- DNA Nucleotides: Include adenine, guanine, cytosine, and thymine.
- RNA Nucleotides: Include adenine, guanine, cytosine, and uracil, differing only in the base.
Understanding these structural elements is vital. They not only clarify how genetic information is stored and transmitted but also highlight the relevance of this conversion in modern biological research.
The Process of Transcription
Transcription is a critical biological process that facilitates the conversion of DNA sequences into RNA sequences. This process is essential for gene expression, as it allows the genetic information encoded in DNA to be transcribed into a working copy of RNA, which can then be translated into proteins. Understanding the process of transcription is paramount for students, researchers, and professionals, as it lays the foundation for many advanced topics in molecular biology, genetics, and bioinformatics.
The significance of transcription cannot be overstated. It serves as the first step in the central dogma of molecular biology, which describes the flow of genetic information from DNA to RNA to protein. This pathway is crucial for virtually all cellular functions. In addition, the regulation of transcription can determine not only when a gene is expressed but also the level of expression, significantly impacting cellular function and organismal development.
When studying transcription, it is important to consider several key elements:
- RNA Polymerase: This enzyme is central to the transcription process, as it synthesizes RNA strands by copying the coding DNA template.
- Promoters: Regions of DNA that provide a binding site for RNA polymerase, initiating the transcription process.
- Regulatory Sequences: DNA segments that can enhance or repress transcription, influencing gene expression.
Recognizing transcription's role in genetic disorders and diseases highlights its importance in biotechnology and medical research. Advances in understanding transcription have led to innovations in genetic engineering and therapeutic approaches to treat genetic disorders.
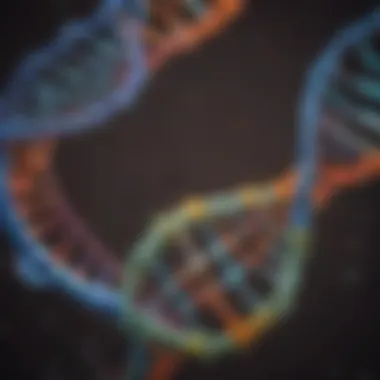
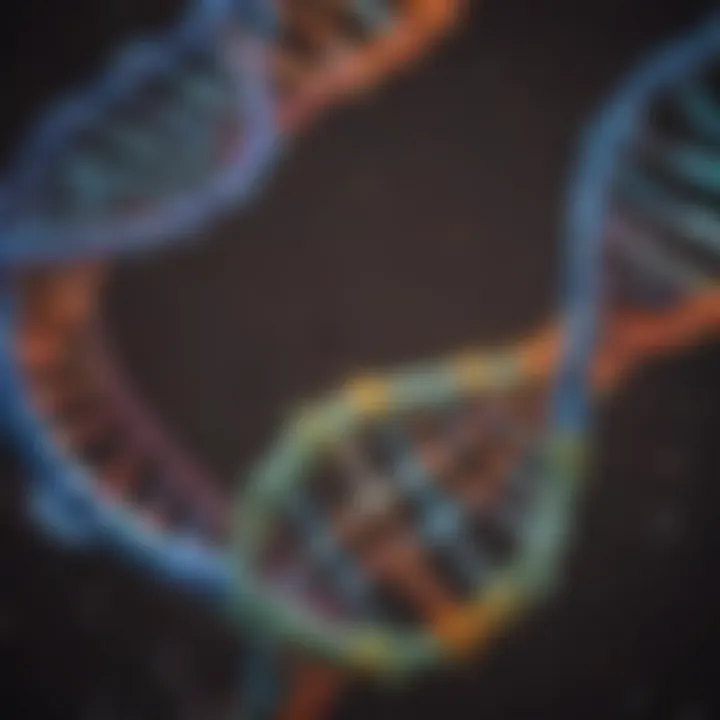
"Transcription is the gateway to understanding gene regulation and expression, offering insights into how genetic information drives biological function."
Initiation of Transcription
The initiation phase of transcription represents a series of carefully orchestrated events leading to the successful binding of RNA polymerase to the DNA template. This begins at a specific location on the DNA known as the promoter. Promoters contain distinct sequences that are recognized by transcription factors. Transcription factors are proteins that mediate the binding of RNA polymerase, facilitating the formation of the transcription initiation complex.
Specific sequences called enhancers can also interact with transcription factors, increasing the likelihood of RNA polymerase successfully binding to the promoter. Once everything is in place, RNA polymerase unwinds a short stretch of the DNA double helix, creating a transcription bubble. This marks the transition from initiation to the next stage of elongation.
Elongation and Termination
Following successful initiation, the transcript elongation process begins. During elongation, RNA polymerase travels along the DNA template, synthesizing a single strand of RNA. This RNA strand is complementary to the DNA template and is synthesized in the 5' to 3' direction. It is important to highlight that RNA polymerase does not require a primer for synthesis, which is a key difference from DNA replication.
As RNA polymerase continues down the DNA, it catalyzes the complementary base pairing. For example, where there is adenine in the DNA template, uracil is incorporated into the RNA strand. This process continues until a termination signal is reached.
Termination involves sequences known as terminator sequences, which signal to RNA polymerase to stop transcription. Upon reaching these signals, the RNA strand is released, and RNA polymerase disassociates from the DNA template. This concludes the transcription process and results in a mature RNA transcript ready for further processing and eventual translation into proteins.
Understanding these phases of transcription is fundamental in molecular biology due to their implications for gene expression regulation and the practical applications in genetic research and biotechnology.
Mechanisms of DNA to RNA Conversion
Understanding the mechanisms involved in converting DNA sequences to RNA sequences is pivotal in molecular biology. This process known as transcription is the first step in gene expression. By exploring these mechanisms, researchers and practitioners can better comprehend how genes are regulated and expressed in various biological contexts. This section dissects the critical components involved in this conversion process.
Role of RNA Polymerase
RNA polymerase is a fundamental enzyme responsible for synthesizing RNA from a DNA template. It plays a vital part in the transcription process. First, it binds to the promoter region of a gene, signaling the starting point for transcription. There are three main types of RNA polymerase in eukaryotic cells: RNA polymerase I, RNA polymerase II, and RNA polymerase III. Each type has a specific function regarding which RNA molecules they synthesize.
- RNA polymerase I synthesizes ribosomal RNA (rRNA), excluding 5S rRNA.
- RNA polymerase II is responsible for creating messenger RNA (mRNA) and some small nuclear RNAs (snRNA).
- RNA polymerase III synthesizes transfer RNA (tRNA) and 5S rRNA.
During transcription, RNA polymerase unwinds the DNA strand, allowing it to read the template strand to construct a complementary RNA strand. This process is highly regulated and can be influenced by numerous factors.
"RNA polymerase acts as a catalyst, transforming genetic information from DNA to an RNA format, necessary for protein synthesis."
Promoters and Regulatory Sequences
Promoters play an essential role in the regulation of gene expression by providing a specially conserved genetic signal that indicates where RNA polymerase should initiate transcription. The sequences located at the promoter region can vary greatly in complexity across different organisms.
Promoters typically consist of:
- Core promoter: Contains essential sequences for the binding of RNA polymerase and initiation of transcription.
- Proximal promoter elements: These sequences can enhance or inhibit the activity of RNA polymerase.
- Distal regulatory elements: These include enhancers and silencers that operate far from the promoter that can modify transcription levels drastically.
In addition to promoters, transcription factors also contribute significantly to the efficiency and timing of RNA synthesis. Transcription factors bind to specific DNA sequences, affecting the recruitment of RNA polymerase to gene promoters.
In summary, the mechanisms of DNA to RNA conversion involve proteins and signals that facilitate transcription, ultimately determining which genes are expressed at any given time. Understanding these mechanisms gives insight into genetic regulation and expression, which is particularly useful in areas such as genetic research and biomedicine.
DNA Sequence Input Formats
Understanding the various DNA sequence input formats is essential for the accurate conversion of DNA to RNA sequences. The choice of format can influence the efficiency and success of the conversion process. Different applications and tools may require specific formats, making familiarity with them crucial for researchers and bioinformaticians.
Properly formatted DNA sequences allow software to accurately interpret the data, minimizing errors. For example, inputting data in the correct format ensures that algorithms effectively apply transcription rules, leading to reliable RNA output.
Additionally, awareness of the formats can guide users in selecting the right tools and software. Each format has its own characteristics and advantages. For instance, FASTA format is widely recognized and suitable for various bioinformatics applications, while GenBank provides rich annotation with structural information. This knowledge can enhance workflow and improve data management in genetic analysis.
Common Formats for DNA Sequences
Several common formats exist for representing DNA sequences. Each has unique features tailored to specific uses:
- FASTA: A simple and widely used format that contains the sequence in a plain text structure. It typically includes a header line starting with "" followed by the sequence.
- GenBank: A format that provides extensive metadata about sequences, including annotations, features, and references. It is more complex than FASTA but is beneficial for comprehensive data.
- FASTQ: Primarily used in sequencing applications, this format includes not only the sequence but also quality scores for each base, making it critical for assessing data reliability.
- SAM/BAM: Formats used for storing sequence alignment information. SAM is a text-based format, while BAM is its binary counterpart, which allows for more efficient storage and retrieval.
These formats serve different purposes and selecting the appropriate one is fundamental for ensuring effective data handling in bioinformatics workflows.
Encoding and Representation of DNA Data
Encoding DNA sequences is crucial for their proper representation and analysis. There are specific methods used to represent sequences in computational systems. For example:
- Base Encoding: Each nucleotide (A, T, C, G) is represented by a specific character in data storage. This representation enables software to perform various analyses efficiently.
- Binary Encoding: Some algorithms transform DNA sequences into binary format for rapid processing. This method can improve computational efficiency in large-scale analyses.
Additionally, working with encoded sequences allows scientists to implement various algorithms more effectively. Proper management and encoding of DNA data lead to streamlined analysis and more reliable outcomes in sequence conversion tasks.
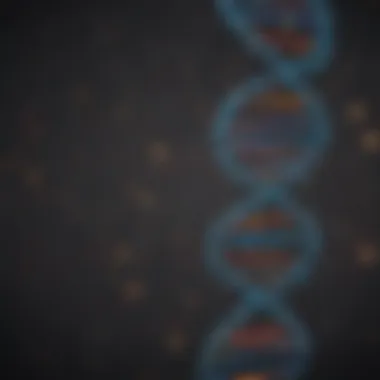
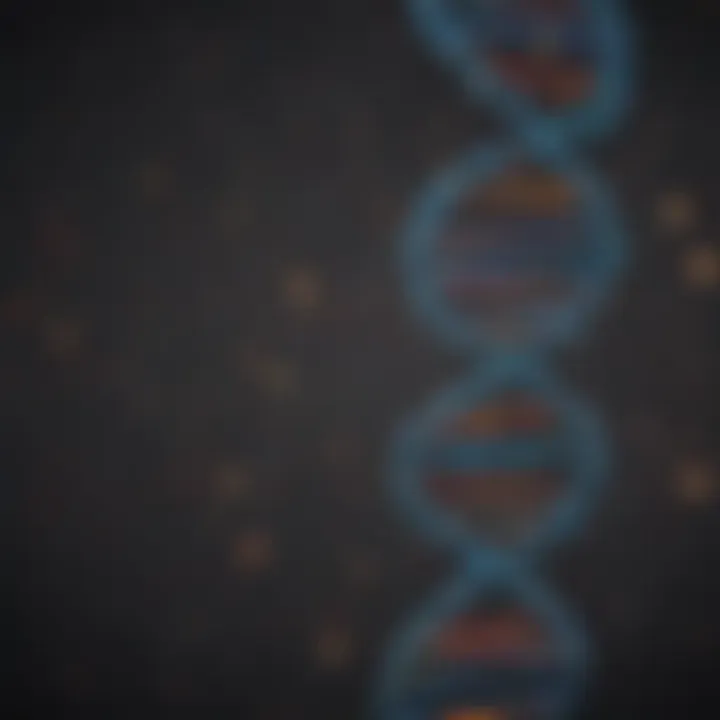
Effective representation of DNA data not only enhances computational processing but also ensures that the biological significance of the data is preserved throughout the analysis.
Familiarity with these encoding methods enables researchers to choose the best approach based on their specific needs, contributing to advancing studies in genetics and molecular biology.
Conversion Algorithms
The transformation of DNA sequences into RNA sequences is not a straightforward procedure. It demands sophisticated methods known as conversion algorithms. These algorithms play crucial roles in bioinformatics, providing the tools to interpret, manipulate, and translate genetic data. The effectiveness of these conversion algorithms can directly impact research outcomes by enhancing the accuracy of RNA sequences derived from DNA.
Utilizing robust conversion algorithms ensures that errors in sequencing data are minimized. This is particularly important for downstream applications in genetic research and biotechnology. Moreover, different algorithms offer diverse benefits, such as speed, scalability, and the ability to handle various data formats, leading to significant efficiency improvements in genomic studies.
Overview of Bioinformatics Tools
Several bioinformatics tools are designed to facilitate the conversion of DNA to RNA. These tools are integral to modern genetic analysis. They aid. Researchers in their tasks by simplifying complex methodologies into user-friendly interfaces. Popular software options include:
- RNAfold: This tool predicts secondary structures of RNA based on sequences. It is essential for understanding RNA folding and function.
- Geneious: A comprehensive software platform that allows for sequence alignment, assembly, and annotation, making it easier to manage genetic data.
- Galaxy: An open-source platform that provides a wide array of bioinformatics tools, including those for DNA to RNA conversion, allowing users to run analyses in a web-based environment.
- SeqMonk: This software specializes in the analysis of sequencing data, enhancing the understanding of the patterns in RNA expression.
These tools significantly increase efficiency and offer in-depth insights into the genetic sequences under study. They are essential for anyone involved in molecular biology research or genetic engineering.
Comparison of Common Algorithms
When evaluating conversion algorithms, one must consider various factors. Performance, reliability, and specificity are critical aspects to assess. The most notable conversion algorithms include:
- Smith-Waterman Algorithm: Known for its accuracy, this algorithm is effective for local sequence alignment. However, it is time-consuming, making it less suitable for very large datasets.
- Needleman-Wunsch Algorithm: This algorithm is designed for global sequence alignment and is highly efficient, though it may overlook local alignment details.
- BLAST (Basic Local Alignment Search Tool): Useful for rapid searches against large databases, BLAST is often favored for its speed. However, it sacrifices some accuracy for speed.
"The choice of algorithm can influence both the speed and accuracy of DNA to RNA conversion processes, guiding researchers in their analyses."
Choosing the right algorithm depends on the specific requirements of the research project. Each has its pros and cons, making it important to understand the context in which these algorithms are used.
Software for DNA to RNA Conversion
The conversion of DNA sequences to RNA sequences is a fundamental process in molecular biology, crucial for expression of the genes. For anyone working in genetic research, biotechnology, or related fields, the use of software specifically designed for this purpose can greatly enhance accuracy and efficiency. Software tools streamline the conversion process, making it easier for researchers to focus on analysis and interpretation rather than manual conversions that are error-prone.
Popular Software Options
Several software options have gained popularity in the scientific community due to their reliability and efficiency in handling DNA to RNA conversions. Tools like Geneious, CLC Genomics Workbench, and Benchling are prominent choices. Each of these platforms provide various functionalities that cater to different user needs.
- Geneious offers an intuitive interface, making it suitable for both beginners and experienced users. It supports a wide array of file types and features tools for editing and analyzing sequences.
- CLC Genomics Workbench is powerful for processing large datasets. It allows users to perform complex bioinformatics analyses, including alignment and visualization of RNA sequences.
- Benchling is a web-based platform that emphasizes collaboration and ease of use, providing necessary tools for transcription analysis and molecular sequence design.
Using these software options can significantly reduce the time it takes to convert DNA sequences to RNA. Their built-in algorithms are optimized to minimize errors, ensuring higher fidelity in sequence representation.
User-Generated Tools and Scripts
In addition to commercially available software, there are numerous user-generated tools and scripts available for DNA to RNA conversion. Many researchers share their custom scripts on platforms like GitHub and forums such as Reddit. These tools often allow for greater customization, enabling users to adjust algorithms to meet specific needs in their research.
Popular examples include:
- Biopython: A collection of scripts that allow users to manipulate biological data, including DNA and RNA sequences. It offers simplicity and flexibility for those comfortable with Python coding.
- Galaxy: An open-source platform that allows researchers to create workflows to analyze biological data. Users can develop their RNA conversion pipelines tailored to specific projects.
- Custom Bash Scripts: Many researchers develop scripts in Bash to automate the conversion process, particularly when handling large quantities of data.
These user-generated tools highlight the collaborative spirit in scientific research. They allow for innovative approaches to solving complex problems, and they often evolve as more users contribute to their development.
"The landscape of bioinformatics tools is continuously evolving, reflecting the dynamic nature of research itself."
Applications of RNA Sequences
The conversion of DNA sequences to RNA sequences plays a vital role in various fields, particularly in genetic research and biotechnology. Understanding the applications of RNA sequences is fundamentally important. RNA serves not only as a messenger between DNA and proteins, but also as a tool for manipulating genetic expression. With the advancements in technology and bioinformatics, applications of RNA sequences have expanded significantly.
Implications in Genetic Research
RNA sequences are critical for advancing genetic research. They enable scientists to study gene expression, regulation, and the effects of mutations. By analyzing RNA sequences, researchers can assess which genes are active under certain conditions. This information is essential for identifying potential genetic disorders and understanding the mechanisms behind them.
- Gene Expression Studies: RNA gives insight into how genes are expressed during different developmental stages or environmental conditions.
- Disease Mechanisms: RNA analysis helps to uncover pathways involved in diseases, opening potential avenues for targeted therapies.
- Functional Genomics: Understanding RNA helps in identifying gene functions and interactions, which is fundamental for new discoveries in genetics.
Furthermore, developing RNA-based technologies such as CRISPR-Cas9 relies heavily on accurate RNA sequences for efficient gene editing. This connection underscores the significance of RNA in furthering the realm of genetic research.
Biotechnology and Medicine Applications
In biotechnology and medicine, RNA sequences have numerous applications that can alter therapeutic approaches dramatically. One primary application lies in the development of RNA-based therapies, particularly those targeting viral infections and genetic diseases. The following highlights important applications:
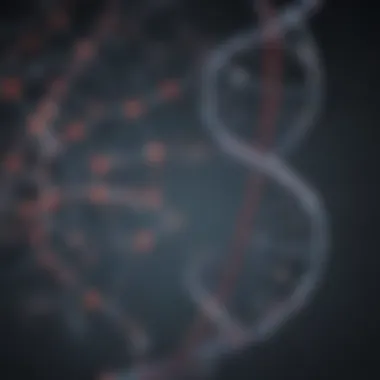
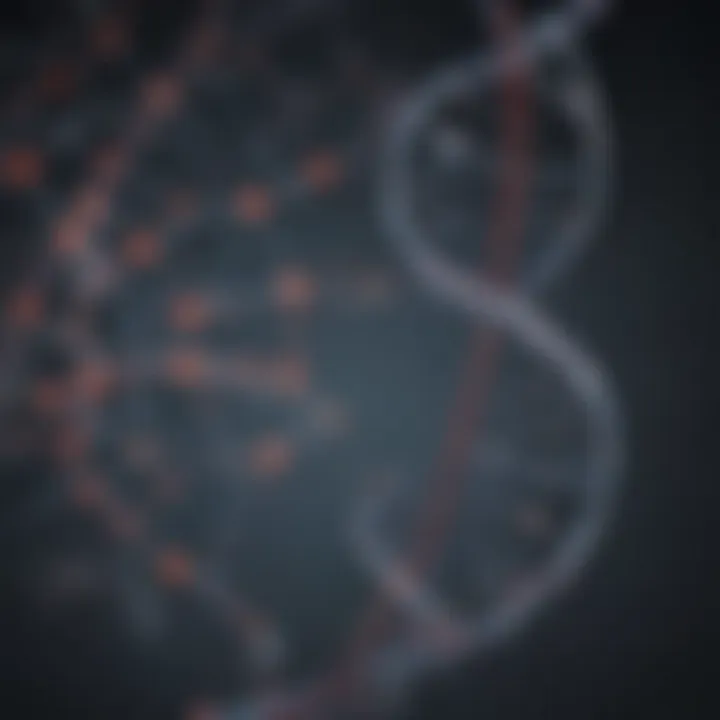
- RNA Interference (RNAi): This technique allows for silencing specific genes, which makes it a promising tool for treating diseases by lowering the production of harmful proteins.
- mRNA Vaccines: As demonstrated by the recent COVID-19 vaccines, messenger RNA (mRNA) vaccines stimulate immune responses without using live pathogens. This technology represents a breakthrough in rapid vaccine development.
- Diagnostic Tools: RNA sequences serve as biomarkers in diagnosing certain diseases, enabling early detection and personalized medicine approaches.
"RNA sequencing technologies are leading the way in transforming diagnostics and treatment strategies in numerous diseases."
The potential contributions of RNA sequences extend from laboratory research to clinical applications, showcasing versatility that significantly benefits both fields of science and healthcare.
In summary, the implications of RNA sequences in genetic research and their plethora of applications in biotechnology and medicine underpin their essential role in contemporary science. As research continues to evolve, understanding and leveraging RNA's capabilities will remain fundamental.
Challenges in DNA to RNA Conversion
Converting DNA sequences to RNA sequences is a critical aspect of molecular biology. Despite its significance, the process is fraught with challenges that can impact both the accuracy and efficiency of the conversion. Identifying these challenges is essential for researchers and practitioners alike, as it helps in understanding the complexities involved and in improving the methodologies used. Among these challenges, issues related to sequence quality and alignment difficulties are particularly noteworthy.
Issues with Sequence Quality
Sequence quality refers to the accuracy and reliability of the DNA sequences prior to conversion. Poor sequence quality can lead to erroneous RNA products, which in turn may impact subsequent biological functions or experimental outcomes. It is crucial to assess sequence quality carefully, as factors such as sequencing errors, contaminants, and inadequate library preparation can degrade the genomic data.
Researchers often utilize metrics such as the Phred score to evaluate sequence quality. High-quality sequences typically exhibit a Phred score greater than 20, indicating a 99% accuracy in base calling. However, obtaining high-quality sequences is not always feasible. In cases where data integrity is compromised due to these factors, the challenges become amplified.
To combat these quality issues, various bioinformatics tools have emerged. Tools like FastQC can provide comprehensive quality checks, enabling researchers to filter and trim low-quality sequences before conversion. Employing these tools is vital, as they help to ensure that the DNA sequences fed into the conversion algorithms are of adequate quality, ultimately leading to more reliable RNA outputs.
Alignment and Mapping Challenges
Once DNA sequences are converted to RNA sequences, the next step often involves mapping these sequences back to a reference genome or transcriptome. Alignment is a process that determines the best fit for RNA sequences compared to known genomic data. However, alignment presents its own set of challenges, primarily due to the inherent complexity of genomic structures.
For instance, alternative splicing—where a single gene can produce multiple RNA variants—complicates the alignment process. Variants can lead to mismatches, making it difficult for algorithms to accurately position the RNA sequences. Moreover, the presence of repetitive regions in the genome can obscure alignment results, as repeats can confuse the mapping algorithm.
Addressing these challenges requires robust alignment tools such as STAR or HISAT2, which are specifically tailored for high-throughput RNA sequencing data. These tools incorporate sophisticated algorithms that can accommodate gaps and mismatches during the alignment process, improving the overall mapping accuracy.
Ultimately, both sequence quality and alignment challenges represent significant hurdles in converting DNA to RNA. Overcoming these challenges is essential for accurate and meaningful biological insights. Understanding and addressing these issues can enhance the reliability of RNA sequences generated from DNA, subsequently maximizing the utility of the data in research and application.
Future Trends in Sequence Conversion
The pursuit of converting DNA sequences to RNA sequences is evolving. As our understanding of molecular biology deepens, we must also consider the technologies and methodologies that are reshaping this field. Future trends in sequence conversion will lead not only to enhanced accuracy but also efficiency in processing vast amounts of genomic data. These advancements could have significant impacts on genetic research, biotechnology, and medicine.
Advancements in Computational Techniques
Computational techniques are transforming how we analyze genetic sequences. Innovations in algorithms have allowed for faster processing of DNA and RNA sequences. For instance, improvements in alignment algorithms are crucial for ensuring that sequences are compared accurately. More efficient usage of computational resources has also become central to handling big genomic data sets. This is particularly vital as the amount of biological data generated continues to grow exponentially.
One notable advancement is the development of parallel computing methods. These methods make it possible to run multiple calculations simultaneously, which can significantly speed up the processing time for complex conversions. Additionally, cloud computing resources enable researchers to access powerful computational tools without the need for extensive local infrastructure.
- Key benefits of these advancements include:
- Increased speed in data analysis
- Enhanced accuracy in sequence alignment
- Improved accessibility to computational power
Integration of AI in Bioinformatics
Artificial intelligence (AI) is becoming a game-changer in bioinformatics. The integration of AI algorithms into sequence conversion can enhance the accuracy and speed of the processes involved. Machine learning models can learn from large datasets, identifying patterns and relationships that traditional methods might miss. As a result, AI could help refine transcription predictions and provide more reliable conversions from DNA to RNA.
For example, AI-powered tools can dynamically adjust their algorithms based on existing data, becoming increasingly effective over time. This adaptability allows for continual improvements to be made in how conversion processes are executed.
"AI's capability to process and analyze biological data is revolutionizing genetic research, providing tools that can identify potential issues early on in the sequencing process."
To summarize, the future of DNA and RNA sequence conversion is poised to benefit significantly from advancements in computational techniques and the integration of AI in bioinformatics. As these tools evolve, researchers will have the means to conduct more detailed analyses. The implications for genetic research and applications in biotechnology and medicine will be profound, enhancing our overall understanding of genetics and its practical applications.
Ethical Considerations
The discussion of ethical considerations serves as a crucial underpinning of the conversation surrounding the conversion of DNA sequences to RNA sequences. As scientific advancements in genetics continue to unfold, it becomes increasingly important to navigate the ethical landscape that accompanies these developments. This section highlights two primary themes: the ethics of genetic manipulation and the responsible use of genetic data.
Ethics of Genetic Manipulation
Genetic manipulation invites a range of ethical dilemmas. At its core, it raises questions about the extent to which humans should interfere with natural biological processes. While the benefits of manipulating DNA and RNA can be profound, such as advancing medical treatments and improving agricultural yields, it also poses potential risks to human health and biodiversity.
One of the significant concerns involves the possibility of unintended consequences. Changes made to genetic structures might lead to unforeseen ecological impacts or health issues. The precautionary principle suggests that these risks be carefully assessed before any significant manipulative actions are taken.
Moreover, there are societal implications to consider. The capacity to edit or modify genes can create disparities between those who have access to such technologies and those who do not. This inequality could result in a new form of bio-elitism, thereby exacerbating existing social divides. The ethical framework around this technology must include not only the scientific aspects but also the societal context in which it operates.
Responsible Use of Genetic Data
As scientists and researchers collect vast amounts of genetic data, the ethical use of this information cannot be overlooked. This data can be extremely sensitive, potentially revealing information about an individual’s predisposition to certain diseases or conditions. Therefore, ensuring confidentiality and privacy in genetic research is paramount. Researchers must adhere to strict guidelines and best practices to maintain the integrity of this data.
Informed consent is also a crucial element of responsible data use. Participants should be fully aware of how their genetic information will be utilized, including any potential for sharing with third parties. The complexities of consent in genetic research highlight the need for transparency and clear communication between researchers and participants.
Furthermore, there is an increasing call for regulations regarding data storage and use. Policymakers must work alongside scientists to create a framework that balances innovation with the protection of individual rights. This collaborative approach can lead to more robust ethical guidelines that not only promote scientific exploration but also safeguard the interests of society.
"The ethical implications of genetic manipulation extend far beyond the lab. They resonate throughout society and demand thorough consideration."